|
Selection Experiments on Patchy Backgrounds
On fine-grained fractal backgrounds, the evolutionary lineages that resulted
from predation by blue jays were far more variable in appearance than
either the non-selected or the frequency-independent controls. So hunting by
searching image clearly increased phenotypic variation.
But at the end of 100 generations of selection, we still did not get a classical
polymorphism. E.B. Ford (1965)
originally defined genetic polymorphism by excluding continuous variation within a single
habitat or discontinuous variation across different habitats. He thereby set limits that artificially excluded a lot of the natural variability of animal coloration.
There are, for example,
a number of species that show "massive" polymorphism, where it is difficult to find
any two individuals in the same population with exactly the same appearance (Whitely
et al. 1997;
Bond & Kamil 2002;
Bond 2007).
And although different color morphs of a single species may be selected in adjacent
contrasting habitats, such divergent forms do not necessarily stay put. Animals
often intermingle across habitat types (Kettlewell 1973; Majerus 1998), creating local
discontinuous polymorphisms that can subsequently be sustained by selective predation.
Color polymorphism is not as simple or as discrete a phenomenon as Ford (1965)
wanted to believe.
There are, however, quite a few species
that are discrete, Fordian polymorphisms, occurring
in a strictly limited number of highly
distinctive morphs, all happily interbreeding with one another in a single local
habitat. These systems are commonly maintained by genetic mechanisms -- sometimes
fairly bizarre
ones -- that suppress intermediate, hybrid forms (Ford 1965), but it is not
clear why such adaptations were selected in the first place, or which environmental
conditions guided their initial evolution. In particular, why do such species have just
that particular number of morphs and not more or less? Why do little nymph underwing
moths (Catocala micronympha) show up in at least nine different forms (there may
actually be dozens), while relict underwings (Catocala relicta) make do with only
five (Barnes & McDunnough 1918)? Why do grouse locusts (Acrydium arenosum) have
twenty distinctive morphs (Nabours et al. 1933) while wrangler grasshoppers
(Circotettix rabula) occur only in either red or gray-green forms (Gillis 1982)?
The number of morphs in a given species
could, of course, be an evolutionary accident. Genetic mechanisms that prevent morph
hybridization vary a lot across species and taxonomic groups, suggesting that
they could have evolved as independent accidents and were retained more because they
provided protection against hunting by searching image than because they produced a
particular suite of color patterns. But it seems likely that the patchiness or
granularity of the visual environment also played a role. For most prey species, the
environment is heterogeneous, a mosaic of patches with contrasting
distributions of color or pattern (Endler 1990). Because camouflage depends on achieving a
sufficient resemblance to the background, these disparate patches effectively form
ecological niches, distinctive coloration regimes to which the
appearance of the prey can be adapted. Under some circumstances, such heterogeneous
environments should promote disruptive selection,
generating polymorphic specialists adapted to each of the available patch types (Merilaita
et al. 1999). Theory predicts that disruptive selection will vary with what Levins (1968)
called the “grain” of the habitat. Habitat grain is a function of the size of patches
relative to the size and mobility of the prey organism. In coarse-grained habitats,
many individuals may spend most of their time -- and experience most of their risk
of predation -- in only one patch type. This environment will select for ecological
specialists, like the wrangler grasshoppers whose red and green dimorphism mirrors
the patches of red granite and gray-green sagebrush in the high desert of Colorado
(Gillis 1982). Fine-grained habitats, in which most individuals experience selection
on many differently colored patches, will select for generalists that are roughly equally
detectable on all patch types (Levins 1968; Kisdi 2001).
To investigate the effects of habitat
grain, we made use of our “virtual ecology” system. As in our earlier study with uniform
backgrounds
(Bond & Kamil 2002),
there were two fields of fractal texture shown in each predation trial. Three squads of
six jays each were tested in three different operant chambers. Each bird received
a series of 160
predation trials per day. On half of the trials, one moth was placed in a randomly chosen
position in one of the two fields of cryptic background. On the remaining, negative trials,
only the background fields were shown. The backgrounds were mixtures of dark and light
colored pixels at three different spatial scales
(Bond & Kamil 2006).
Half of the pixels in the display fields were drawn from each of two contrasting
generating distributions, producing light and dark textured patches. Depending
on the experimental treatment, the patches were at different scales of
heterogeneity.
Moths were placed at random on the
background fields in each trial, so the impact of patchiness on selection was not a result
of maintaining separate dark and light breeding populations. Like peppered moths
(Majerus 1998), our virtual insects reassorted themselves during the night and did not
choose particular matching backgrounds to land on, and they made no distinction between
the colors of moths they mated with. This is realistic, but it also reduces the intensity
of selection for background matching. None of our backgrounds were truly "coarse grained,"
in Levins' (1968) sense. Patchiness could only affect prey polymorphism through its
influence on searching image.
|
A set of four moths -- two
light colored and two dark colored -- are shown on the left on the three experimental
treatments: In the Disjunct treatment (a), each entire background
field was drawn from one of the two distributions, creating patches that were 15
times the size of a moth. In the Mottled treatment (b), the two
distributions were coarsely mixed across both fields, resulting in patches that
were about the same size as a moth. In the Speckled treatment (c),
the two distributions were finely intermixed, resulting in patches that were 1/12th
the size of a moth.
|
In all of
the experimental replications, the resulting pattern of selection faithfully
reflected background differences in both patch size and texture. To illustrate, the
graphs below show phenotypic spreads of moth populations from the 100th
generation of selection for three lineages, one from each treatment type. Each
moth image is placed at a point along the horizontal axis that corresponds to its
average brightness, in terms of the mean value of its constituent pixels.
So the moths range from nearly black on the left to nearly white on the right.
The degree of dark/light polymorphism was a reflection of the size of the
background patches. The Disjunct treatment, with the largest patches, produced
the sharpest contrast in average brightness -- there were lots of very dark and very
light moths and few intermediate ones. There was a smaller, though still
statistically significant, dark/light polymorphism in the Mottled treatment
with its smaller, moth-sized patches, though the images were more bunched up in the
center of the range, with fewer all white or all black moths.
The average brightness effect in the Speckled lineage was insignificant
-- moths were essentially scattered uniformly across the entire range.
The position of each moth image on the vertical axis indicates the standard
deviation of its pixel values, essentially a measure of the variance of its texture.
So the moths range from a uniform texture of all white, all black, or all gray at
the bottom to a grainy texture of multi-colored pixels at the top. The moth textures
were most uniform for the large patches in the Disjunct treatment, where the pixels
surrounding each moth on its background were always about the same coloration. Moths
in the Mottled treatment had more variable textures, because they often landed on
edges between dark and light patches. In the Speckled treatment, the moth images
were always surrounded with a wide range of background pixel values, insuring that
they would evolve a much more variable and complex appearance.
As expected from ecological theory, larger habitat patches produced stronger
differences between a limited number of morphs, while small patches produced
a less distinctive, more continuous polymorphism, closer to what has been
described in beach clams (Donacilla cornea;Whiteley, et al. 1997) or
army cutworms (Agrotis infusa, Common 1954). What is striking, however,
is that this effect occurred even when the moths were placed in wholly random
positions on the background. Dark and light morphs
in the Disjunct treatment were not the product of strong disruptive selection;
they did not exclusively live and breed on a specific background type. Apparently,
when the background consists of very large, very discontinuous color patterns,
the blue jays adopt a search strategy that effectively imposes a weak disruptive
selection even on randomly distributed prey items. To understand how this
worked, we analyzed the data for indications of hunting by searching image.
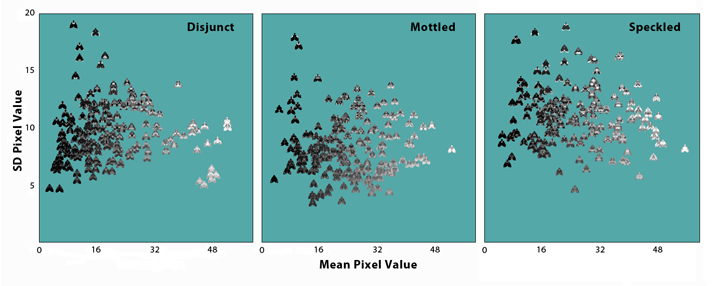
Evidence for Searching Images
As in our earlier study
of selection on a uniform background
(Bond & Kamil 2002),
we analyzed for searching image effects using the relationship between how accurately
a target moth was detected and how similar it was to the most recent previously
detected moth. If detection accuracy was higher when successive moths looked
a lot alike and lower when they were very different, the best inference is that the
previous detection had "primed" the blue jay to attend to that particular pattern.
So if the next moth was similar in appearance, the bird would be attending to that
stimulus configuration and would be much more likely to find the moth. If the next
moth was very different, the bird's attention would still be focused on the previous
moth -- it would be looking for something totally different and would not readily locate
the new moth.
This suggests that detection accuracy should be high when successive
stimuli are similar and should decrease significantly when they are different.
If, on the other hand, the sequence of moth targets had no effect on
how well the birds were able to find them, accuracy should be about the same whether
the previous moth was similar or different. This result would suggest that the blue
jays were not using searching images to locate the moth targets (at least in that
experimental treatment). The attentional priming effect is, thus, the cleanest
quantitative test for the use of searching images by visual predators
(Bond & Kamil 2006,
Kamil & Bond 2006,
Bond 2007,
Goto et al. 2014).
Each small panel in the graph below shows detection accuracy (along the Y axis, from
0 to 1) on blocks of 100 trials as a function of the similarity between the target
and the last previous correctly detected moth (along the X axis, from high to low
similarity). In these plots, searching image effects appear as a negative regression
slope, a downward trend along the X axis, indicating higher accuracies for moths
that were more similar to previously detected targets. The crypticity of the target
moth increases from the bottom to the top panel, dividing the range of crypticities
within each treatment column into percentile groupings: Low (0 to 33rd), Medium
(34th to 66th), and High (67th to 100th). Background patchiness increases from the
left panels (A1-A3) to the right ones (D1-D3), ranging from the uniform texture used
in Bond and Kamil (2002) to the three treatments used in Bond and Kamil (2006).
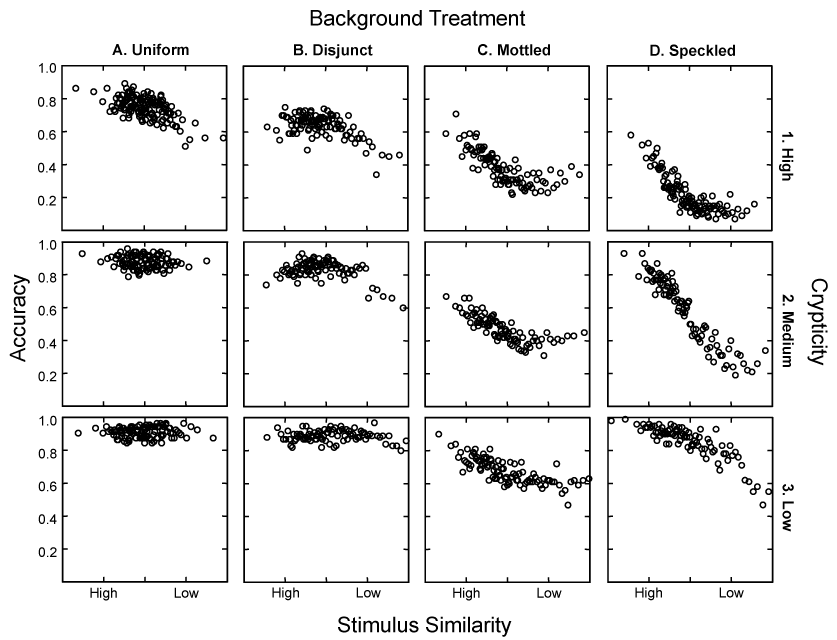
Across the whole range of treatments,
detection accuracy was uniformly greater for low crypticity targets (row 3) than for
moderately cryptic ones (row 2), and greater for moderately cryptic targets than for
highly cryptic ones (row 1). This confirms that our crypticity measure provides
a realistic sense of task difficulty. It is also clear that finer grained backgrounds
tend to interfere with moth detection: Accuracy levels for columns C and D were on the
average lower at each crypticity level than those for columns A and B. But the most
striking effect was that the slope of the relationship between accuracy and stimulus
similarity changed systematically with background treatment. Significant searching
image effects were displayed in high crypticity moths on the Uniform and Disjunct
backgrounds (A1 and B1) and in all crypticity groupings on the Mottled and Speckled
backgrounds (C1-C3 and D1-D3). Hunting by searching image was mainly displayed on
fine-grained backgrounds, where the resulting apostatic selection produced a very
diverse collection of moth images and little or no evidence of discrete polymorphism.
On the disjunct background, in contrast, the birds were minimally affected by the
appearance of the previous target. Instead, the results suggest that they simply
scanned each display looking for local irregularities in the background pattern.
When they found an anomalous spot, they pecked it. And because the anomalies were
usually due to the presence of a moth, the birds were rewarded for this more global
response strategy. In fact, they were probably not searching for moths, as such, at all
on the disjunct backgrounds. They were probably restricting themselves to scanning
for pretty much any deviations in color and texture. So the primary inference from
our study of background heterogeneity was that the strongest indications
of evolved prey dimorphism occurred on backgrounds where searching image effects
(with their promotion of apostatic selection) were not particularly involved.
In summary, apostatic selection due to searching image effects works
to maintain polymorphism, but it does so most effectively on backgrounds where all
moth types are fairly hard to find. The result is a continuous, massive polymorphism.
Where the environment is divided into highly contrasting dark and light patches, a discrete
polymorphism develops -- as E.B. Ford would have predicted -- generating a
population that consists of just light and dark moths. But the selection process
does not rely on finely detailed searching images. The birds simply wipe out any
clearly discrepant moth on both backgrounds. Apostatic selection among discretely
distinctive moths apparently only occurs when the morphs are fixed in appearance and
intermediate forms cannot occur, as in
(Bond & Kamil 1998).
Additional discussion of this issue can be found in our review articles
(Kamil & Bond 2006 and
Bond 2007
), and the whole concept of visual search and prey evolution is placed in a broader
context in our book,
Concealing Coloration in Animals,
published in 2013 by Harvard U. Press.
References from Other Sources
Barnes, W, & McDunnough, J. (1918). Illustrations of the North American species of the genus
Catocala. Memoirs of the American Museum of Natural History, New Series 3,
Part 1.
Common, I.F.B. (1954). A study of the ecology of the adult bogong moth,
Agrotis infusa (Boisd.) (Lepidoptera: Noctuidae), with special reference to its
behaviour during migration and aestivation. Australian Journal of Zoology
2: 223-263.
Endler, J.A. (1990). On the measurement and classification of colour in studies of animal
colour patterns. Biological Journal of the Linnean Society 41:
315-352.
Ford, E.B. (1965). Genetic Polymorphism. London: Faber & Faber.
Gillis, J.E. (1982). Substrate colour-matching cues in the cryptic grasshopper
Circotettix rabula rabula (Rehn & Hebard). Animal Behaviour
30: 113-116.
Kettlewell, H.B.D. (1973). The Evolution of Melanism. Oxford: Clarendon
Press.
Kisdi, É. (2001). Long-term adaptive diversity in Levene-type models.
Evolutionary Ecology Research 3: 721-727.
Levins, R. (1968). Evolution in Changing Environments. Princeton: Princeton
Univ. Press
Majerus, M.E.N. (1998). Melanism: Evolution in Action.
Oxford: Oxford Univeristy Press.
Merilaita, S., Tuomi, J., & Jormalainen, V.(1999). Optimization of cryptic coloration
in heterogeneous habitats. Biological Journal of the Linnean Society
67: 151-161.
Nabours, R.K., Larson, I., & Hartwig, N. (1933). Inheritance of color patterns in the
grouse locust Acrydium arenosum Burmeister (Tettigidae). Genetics
18: 159-171.
Whiteley, D.A.A., Owen, D.F., & Smith, D.A.S. (1997). Massive polymorphism and natural
selection in Donacilla cornea (Poli, 1791) (Bivalvia: Mesodesmatidae).
Biological Journal of the Linnean Society 62: 475-94.
|