|
Selection Experiments on Uniform Backgrounds
We conducted three
successive runs of a selection experiment on our uniform fractal background
(Bond & Kamil 2002),
in which the effects of selection
within experimental lines were contrasted to those in control lineages
exposed to differing selective regimens (Kassen 2002). Each time, we
began with the same parental population and continued predatory selection
out to the 100th generation of progeny. Between runs, the jays were given
30 days of exposure to the parental population under stationary,
non-evolving conditions to return them to a consistent baseline.
For each moth,
we recorded whether it was detected by the jay and, if so, how long
the bird took to find it. In addition, we estimated the resemblance
of each moth to the background, using our crypticity index, and
determined its phenotypic disparity from the rest of the prey population.
To quantify disparity, we applied a non-parametric partitioning
algorithm (Kaufman & Rousseeuw 1990) to the moth phenotypes
in each generation, choosing a prototypical individual with minimal
average distance from all others in phenotypic space. This "taxonomic
distance" (Sneath & Sokal 1973) for each moth expressed the
magnitude of its deviation from the prototype, and the average distance
over all moths in each population provided an estimate of phenotypic variability.
To test whether
observed changes in crypticity and phenotypic variance in the experimental
lines were statistically meaningful, the results were contrasted
with those from two sets of control lineages, one employing no selective
agency and the other using frequency-independent directional selection
based on parameters derived from global aspects of the jays' behavior.
In both of the control treatments, we used the same population size,
the same initial parental population, the same backgrounds, and
the same mutation rate as in the experimental trials. In the non-selected
lineages, however, the moths were never presented to jays, and the
probability of being chosen to breed was uniform across the moth
population, irrespective of phenotype. This provided a control for
the occurrence of directional selection for crypticity in the experimental
treatments.
The second control
was designed to assess our primary hypothesis: that frequency-dependent
selection resulting from hunting by searching image promotes increased
phenotypic diversity. This required comparison to lineages in which
selection was independent of the frequency of particular phenotypes,
but was otherwise similar in intensity and direction to that produced
in the experimental lines. For these control lineages, therefore,
we determined the functional relationship between detection and
crypticity for the jays, averaging over all of the results in the
experimental lines. This function was then used to determine the
probability of a moth's being chosen to breed.
The jays allocated their
searching effort at least in part on the basis of the average degree of
difficulty they experienced in finding the moths: Cryptic moths were detected
more accurately
and rapidly when the rest of the individuals in the population were
cryptic than when the rest were relatively conspicuous. This
"search rate" (Guilford & Dawkins 1987) or "caution"
(Bond & Riley 1991)
effect has been observed in other experimental studies
of foraging behavior, and although caution cannot produce frequency-dependent
predation when prey are presented in a randomized sequence (Endler
1991), it provides a solid basis for an alternative, frequency-independent
control. The selection algorithm in the frequency-independent lineages
was based on the empirical relationships between crypticity and
detection performance in the experimental trials and fitted to the
observed effects of mean population crypticity. We generated 200
selection lines of 100 successive generations using the non-selected
and frequency-independent control treatments to provide controls
for the selective effects of jay predation.
Crypticity
Effects
Over
successive generations, the experimental moths evolved to become significantly
harder to detect, indicating strong directional selection for increased
crypticity. The graphs below show changes in mean crypticity across
successive generations in the three experimental lines (plotted with
symbols), contrasted with the distribution of values from the two
sets of control lines. Non-selected lines form the control group in
panel a; the control in panel b was produced
by frequency-independent selection. The graphs display medians (solid
red) and 95% confidence limits (dotted) from 200 replicate
control lines. Crypticity increased across generations to some degree
in all three treatments; the increase was greatest for the frequency-independent
controls and least for the non-selected lines.
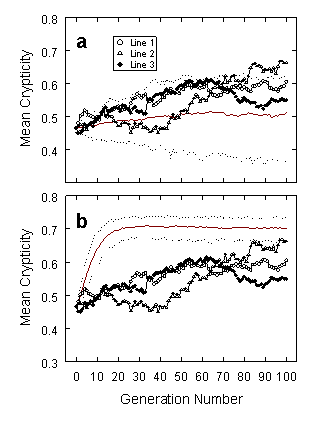
Selection in favor
of individuals that resemble the background has been invoked as
the probable cause of cryptic coloration in prey species for over
a century (Poulton 1890), and there have been numerous demonstrations
that predators preferentially feed on more conspicuous prey items
(reviewed in Endler 1978, Cott 1957, Robinson 1969, and
Diamond & Bond 2013).
Our research is still, however, one of the only studies other than Endler's
(1980) work on color-pattern selection in guppies that has shown significant
directional selection by predators over multiple successive prey generations
when compared to non-selected controls.
Phenotypic
Variance
Our
most important finding, however, was that the experimental lines showed
significantly greater phenotypic variance than either control, demonstrating
that frequency-dependent selection by visual predators can, of itself,
promote high phenotypic diversity in prey species. The graphs below
show changes in phenotypic variance of the digital moth population
in the three experimental lines (plotted with symbols), contrasted
with the distribution of values from the two sets of control lines.
Again, non-selected lines form the control group in panel a,
and frequency-independent lines are shown in panel b.
Graphs display medians (solid red) and 95% confidence limits
(dotted) from 200 replicate control lines. Phenotypic variance
increased to some degree in all three treatments, but the increase
was greater in the experimental lines than in either of the sets of controls.
Experimental lines 1 and 3 each showed a striking, abrupt shift to
a higher level of phenotypic variance at some point in the course
of selection trials. In each case, the shift appeared to have been
produced by the explosive spread of mutant regulatory genes affecting
global levels of brightness or contrast.
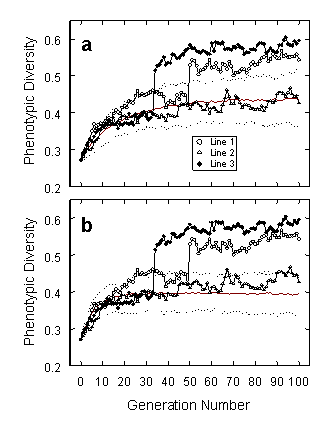
Evidence for
Searching Images
Additional analyses
of the pattern of variation in detection as a function of prey sequences
showed that the primary selective effect was due to jays overlooking
atypical cryptic moths, the most elegant evidence of searching images
that we have encountered and the first demonstration of searching
images when the target stimuli were continuously variable.
|
These graphs
show detection accuracy on blocks of 100 trials as a function
of crypticity of the target moth and dissimilarity between
the target and the last previous correctly detected moth.
Dissimilarity has been adjusted to remove the influence of
mean phenotypic variance and mean population crypticity. Individual
moth crypticity, c, increases from the top to the bottom panel:
Low (c <= 0.45), Medium (0.45 < c <=
0.6), and High (c > 0.6). Regression lines indicate
the relationship between accuracy and dissimilarity within
crypticity levels.
Note that
dissimilarity has no effect on detection accuracy at low or
medium crypticity levels. When the moths are very difficult
to detect, however, the birds are strongly influenced by the
sequence of prey encounters. When two similar, cryptic moths
occur in sequence (low dissimilarity), the birds are much
more accurate than one would expect; when the next moth is
unlike the previous correct detection (high dissimilarity),
however, the birds' performance declines, and they are much
more likely to overlook the moth. This is precisely the pattern
of responses that would be expected from hunting by searching
image.
|
References from Other Sources
Cott, H.B. (1957). Adaptive Coloration in Animals. London: Methuen.
Endler, J.A. (1978). A predator's view of animal color patterns. Evolutionary Biology
11: 319-364.
Endler, J.A. (1980). Natural selection and color patterns in Poecilia reticulata.
Evolution 34: 76-91.
Endler, J.A. (1991). Interactions between predators and prey. In
Behavioral Ecology, J.R. Krebs & N.B. Davies (eds.), pp. 169–96. London:
Blackwell.
Guilford, T, & Dawkins, M.S. (1987). Search images not proven: A reappraisal of
recent evidence. Animal Behaviour 35: 1838-1845.
Kassen, R. (2002). The experimental evolution of specialists, generalists, and
the maintenance of diversity. Journal of Evolutionary Biology 15: 173-190.
Kaufman, L. & Rousseeuw, P.J. (1990). Finding Groups in Data. New York: Wiley.
Poulton, E.B. (1890). The Colours of Animals: Their Meaning and Use, Especially
Considered in the Case of Insects. New York: Appleton.
Robinson, M.H. (1969). Defenses against visually hunting predators.
Evolutionary Biology 3: 225-259.
Sneath, P.H.A. & Sokal, R.R. (1973). Numerical Taxonomy. San Francisco: Freeman.
|